Tucked away in a dusty corner of St. Mary’s Hospital in London lies a tiny, one-room museum dedicated to one of the most important discoveries in the history of medicine: a mold that changed the world. Curators have recreated Alexander Fleming’s laboratory as it would have looked on the day of his discovery, from the cigarettes that he smoked incessantly while working in the lab to a replica of the famous Petri dish of Penicillium.
While samples of the original isolate, known as Penicillium rubens IMI 15378, are cryopreserved in collections around the world, this strain is curiously absent from modern-day commercial penicillin production. The isolate used in mass production today didn’t originate in Fleming’s laboratory at all; instead, the multi-billion dollar industry uses a microbe derived from a moldy cantaloupe found at a fruit market in Peoria, Illinois in the early 1940s.1
While the serendipity of Fleming’s original discovery is widely known, the following lesser-known developments are equally unusual: biographer Gwyn Macfarlane described the process as “a series of chance events of almost unbelievable improbability.”2 These scientific advancements would have immediate impact, preventing death from infection for an uncountable number of soldiers towards the end of World War II, but also long-lasting effects whose repercussions are still felt today.
“Penicillin is, I would argue, the single most important medication that’s ever been introduced,” said Robert Gaynes, an infectious disease physician and medical historian at Emory University. “It completely changed the way we think about drug discovery,” he continued. “It transformed the pharmaceutical industry in many ways, and it completely changed the thinking about treating infectious diseases.”
Penicillin’s Inauspicious Beginnings
Although Fleming’s discovery of Penicillium’s unusual properties is famous today, his 1929 publication made relatively little impact at the time.3 Indeed, said Gaynes, when Fleming presented his study to the Medical Research Club in London, not a single question was asked.
Alexander Fleming first observed the antibacterial effects of Penicillium mold in 1928, but the first attempts to treat humans with penicillin did not occur until more than a decade later.
Ministry of Information Photo Division Photographer
Fleming had observed and carefully noted the effects of the mold on bacteria, but he—along with his increasingly frustrated assistant—was unable to isolate the antibacterial substance itself. Fleming enlisted the help of Harold Raistrick, a biochemist at the London School of Tropical Medicine and Hygiene.4 Yet Raistrick’s team also abandoned the project after a few years, largely due to “the advice of medical friends that penicillin could never be of practical use in clinical medicine because of its instability.”5
Fleming’s work might have been relegated to the scrap heap of history if Ernst Chain, then a biochemist at Oxford University, had not found the 1929 paper nearly a decade later. Chain and Oxford pathologist Howard Florey were instantly intrigued by Fleming’s bacteria-killing mold, and after that, their research proceeded quickly. By 1940, the Oxford research group, which also included biochemist Norman Heatley, had determined how to grow the mold and purify the penicillin, and shown that it could save mice from three different types of deadly bacteria.6
Tests in human patients began almost immediately. On February 12, 1941, researchers administered the first dose of penicillin to a man with a life-threatening Staphylococcus aureus infection.7 After just 24 hours, his condition had improved dramatically, but by February 17, the supply of penicillin had run out. The infection returned and within a month, the patient died.
Researchers knew that the discovery and isolation of this near-miraculous substance would be meaningless if they could not figure out how to scale up its production. But as World War II dragged on and German bombs devastated many major cities, Britain did not have the resources to devote to this massive undertaking.
And so, said Gaynes, “Florey was forced to turn to the United States for help.”
Fleming’s Mold Comes to America
In the spring of 1941, Florey met with Warren Weaver, the director of the Division of Natural Sciences at the Rockefeller Foundation, during Weaver’s visit to London. Florey explained his inability to produce sufficient quantities of penicillin in war-torn Britain. Weaver determined that the Foundation would fund Florey and Heatley’s travel to the US, where they could seek help from government research institutions and pharmaceutical companies to turn their dreams of mass-producing penicillin into a reality. While the US had not yet officially entered WWII, the government had been providing aid to the Allied powers for some time, so Florey gambled that US scientists would be willing to join the cause.
So, in June of that year, Florey and Heatley made their way to the US, bringing with them the precious cultures of Penicillium. Their first visit was to Yale University, where John Fulton, an old friend of Florey’s, was working as a professor. Through Fulton’s scientific connections—and subsequently through a chain of other researchers and government officials—Florey and Heatley made their way to Washington, DC and then to the Northern Regional Research Laboratory (NRRL) in Peoria, Illinois.
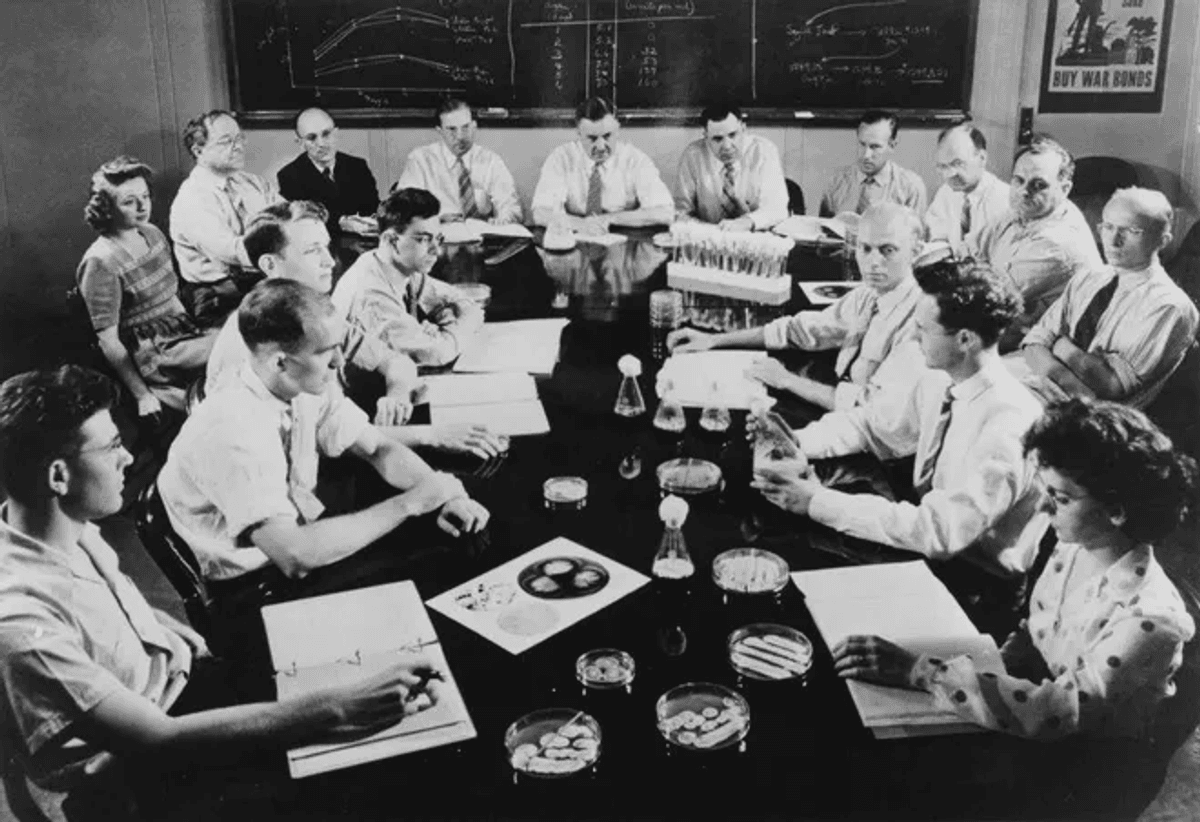
The United States Department of Agriculture’s penicillin research team, which included Dorothy Alexander, Kenneth Raper, Robert Coghill, and Andrew Moyer, meeting in 1944.
United States Department of Agriculture
Robert Coghill, the head of the NRRL’s Fermentation Division, would later note, “Penicillin has often been referred to as a miracle drug, but one of the least understood miracles connected with it is that Florey and Heatley were directed to our Peoria laboratory.”8
Their time in Peoria was short but impactful: the duo shared Fleming’s mold strain and everything they knew about culturing it. After the pair of Oxford researchers returned home in early 1942, the NRRL scientists worked doggedly to improve yields of the drug. Several crucial developments happened in Peoria that enabled penicillin to progress from a substance of the utmost rarity—so rare that early human trials involved harvesting and re-using the penicillin excreted in patients’ urine—to a clinically useful product of global importance.
With years of expertise in fermentation, the NRRL group had developed several techniques to increase the growth of microbes of interest, which they applied to the Penicillium strains.
First, they used different ingredients in their culture media, developed by NRRL mycologist Andrew Moyer. The important ingredient, said Gaynes, was “a byproduct of corn starch production which was called corn steep liquor. It was a waste product. They were lousy with it in the middle of the Midwest. And it turned out that—again, there’s the serendipity—it was much better for growing up the Penicillium than anything that Heatley had used. It was another example of just unbelievable luck.”
Researchers at the time felt similarly. “[The NRRL] was, I am sure, the only laboratory in the country where the corn steep liquor medium would have been discovered,” asserted Coghill. “The discovery of its key place in a penicillin medium was foreordained and inevitable once the problem was assigned to our Fermentation Division.”8
The NRRL researchers also increased yields using a submerged fermentation process, in which the microbes were grown in vessels subjected to constant mixing to aerate them, instead of surface fermentation, in which microbes were grown in large, flat dishes.9
Their lucky streak continued. Coghill, along with Kenneth Raper and Dorothy Alexander, his colleagues in the NRRL Fermentation Division, tested hundreds of Penicillium strains to determine whether any of these had higher penicillin yields than the Fleming strain.10 They gathered an enormous variety of samples, collecting microbes from moldy breads, cheeses, fruits, and vegetables, as well as enlisting the help of the Army Transportation Corps to acquire soil samples from countries around the world, including England, Brazil, Australia, and India. They needn’t have looked so far afield, for, as Coghill noted, “the travesty of this worldwide hunt was that the best producer of all was cultured from a moldy cantaloupe picked up in a Peoria fruit market.”9
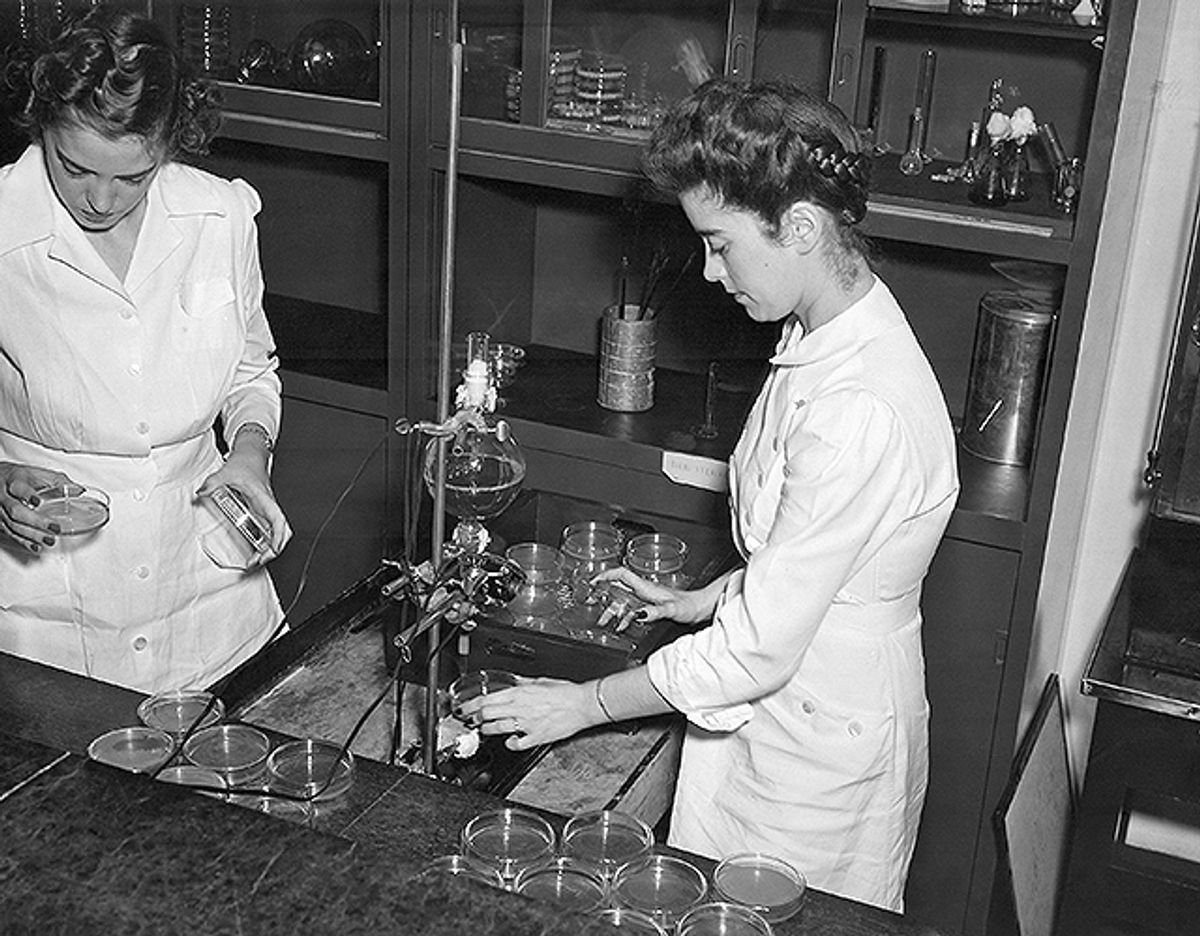
Laboratory technicians helped develop methods for increasing penicillin yields at the Northern Regional Research Laboratory. The woman on the left is believed to be Mary Hunt, who discovered a higher-producing strain of the mold at a Peoria fruit market.
United States Department of Agriculture
There is some controversy over who exactly found the “cantaloupe strain,” or Penicillium rubens NRRL 1951, which is now the state microbe of Illinois. In the decades following the discovery, local newspapers reported that the cantaloupe had been found by NRRL technician Mary Hunt, nicknamed “Moldy Mary,” and indeed, Hunt is listed in the acknowledgements of the 1944 paper on the microbe’s identification.11 Coghill, Raper, and Alexander wrote that “We are likewise indebted to Miss Mary K. Hunt for collecting samples of moldy materials and for assisting in the isolation and preliminary testing of many strains.”10 Years later, however, Raper told a reporter that the cantaloupe had simply been dropped off by a local housewife.9
Regardless of who initially found it, the cantaloupe strain gained great importance as the “parent” from which today’s commercial strains are derived. The significance of these developments in hastening the mass production of penicillin cannot be overstated: “At the beginning of 1942 they didn’t have any [penicillin], and by the end of ‘42 they had enough for the first clinical trial,” said Gaynes. “And by the end of ‘43 they had enough penicillin to treat all of the Allied armed forces… it really was a remarkable transformation.”
Yet the work of the NRRL researchers went largely unrecognized on the world stage; Fleming, Chain, and Florey, on the other hand, were awarded the Nobel Prize in Physiology or Medicine in 1945.
Antimicrobials: The Golden Age and the Rise of Resistance
The following few decades came to be known as the Golden Age of antibiotic discovery.12 Selman Waksman’s work on soil microbiology at Rutgers University led to the isolation of streptomycin, the first antibiotic that was effective against Mycobacterium tuberculosis, in 1943.13 Nine years later, this discovery won him the Nobel Prize. Between 1940 and 1970, researchers discovered more than 20 new classes of antibiotics, most of which were derived from bacteria and fungi.14
But the pace of discovery soon slowed dramatically. Despite major technological advancements, relatively few new antibiotics or antifungals have been introduced in the present century. And while these drugs have largely remained the same, pathogenic microbes—and the planet—are undergoing rapid and profound change.
The pace of microbial evolution should come as no surprise: In the very same 1941 paper in which the Oxford researchers published the miraculous recoveries of patients treated with penicillin, they experimentally demonstrated the alarmingly fast development of antibiotic resistance. They cultured bacteria in gradually increasing concentrations of penicillin and in just a few months noted that “the microbe was able to multiply in a concentration of penicillin a thousand times greater than that which inhibited the parent strain in a parallel test.”7 Today, antibiotic-resistant bacteria kill more than one million people each year; researchers estimate that the annual death toll will nearly double by 2050.15
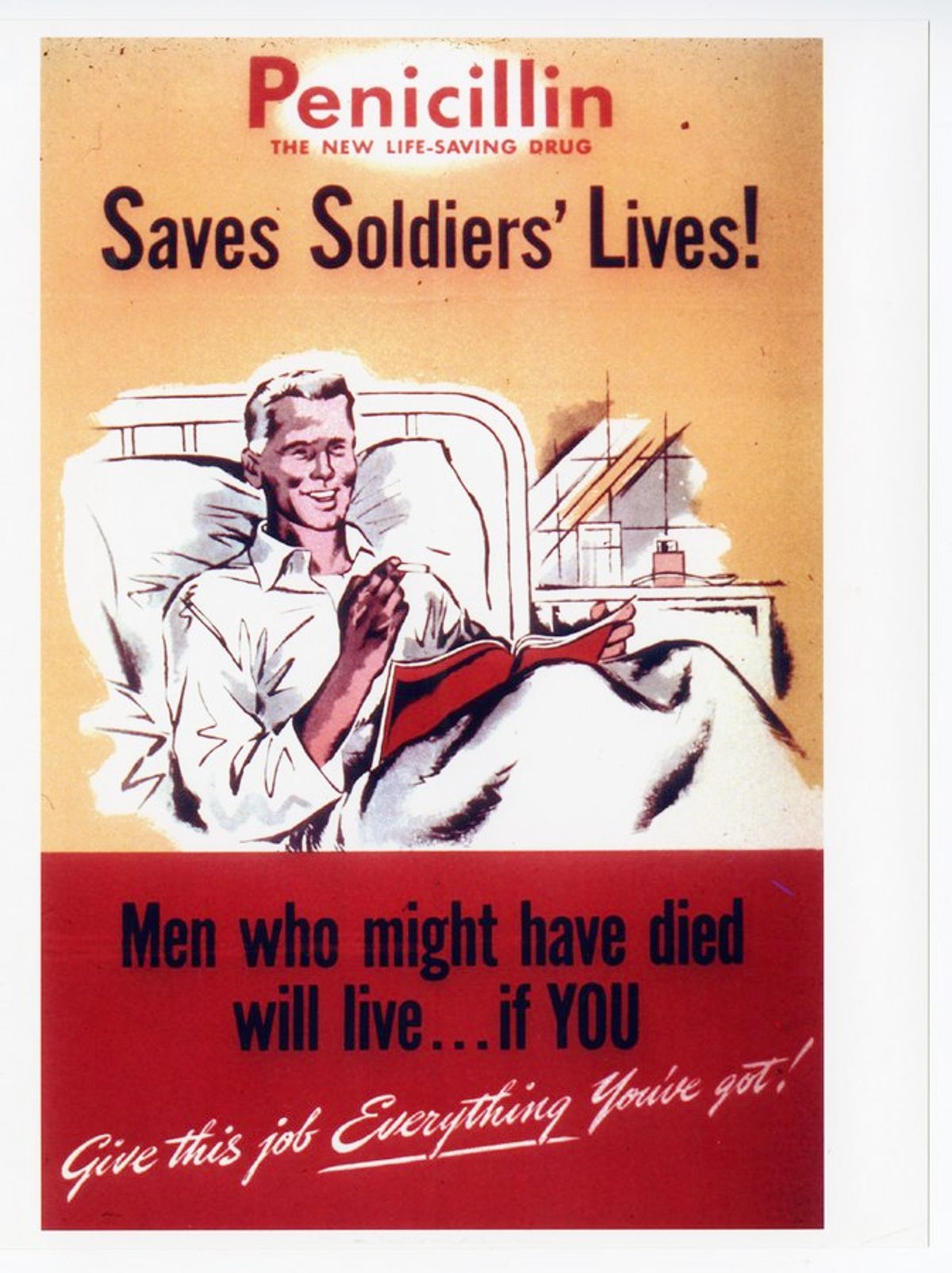
A World War II poster advertises the life-saving capabilities of penicillin.
Science History Institute
Although less well-known, antifungal resistance is also becoming a major threat. Many of the organisms classified as fungal priority pathogens by the World Health Organization are naturally resistant, or have acquired resistance, to at least one of the four classes of antifungals currently in use and researchers have described the rise of pan-drug resistant Candida auris as “alarming.”16,17 Paralleling the development of resistance, and perhaps spurred along by fungal adaptations to a warming climate, global mortality from fungal infections is rising sharply.18,19
As with Fleming’s Penicillium, many researchers are once again turning to fungi for novel antibacterial and antifungal agents. Among them is Nancy Keller, a medical microbiologist at the University of Wisconsin–Madison and cofounder of Terra BioForge. Keller and her team comb through fungal genomes for groups of genes, called biosynthetic gene clusters (BGCs), that could produce useful secondary metabolites, including those that could function as antimicrobials. Given the nature of her work, it is fitting that Keller’s official title is the Robert L. Metzenberg and Kenneth B. Raper Professor of Mycology—the latter being one of the NRRL researchers who was instrumental in the development of penicillin more than half a century ago.
Fungi have been at war with bacteria—and with other species of fungi—for millennia. As such, said Keller, “The metabolites made from these BCGs, a few of them are protective against abiotic stresses like UV… most of them, though, you could think of as weapons or armor, to protect them from other microbes or to defeat other microbes.”
In terms of fungal natural products, Keller noted that most of the low-hanging fruit has already been found, but she believes that many fungal secrets remain to be discovered by looking at these organisms with fresh eyes and new technologies. “If you look at the genome of a filamentous fungus…you can predict that the fungi could make 50 or even 70 metabolites, and we only know a very few of them for each fungus,” said Keller. “So, I personally feel we can get a lot more data even from the fungi around us.”
As the cost of genome sequencing has fallen, more and more fungal genomes have been published. But sorting through reams of genomic data to identify BCGs that could produce novel metabolites is no easy task. To aid in this process, Keller’s group and other research teams have been developing increasingly sophisticated bioinformatic algorithms.20,21
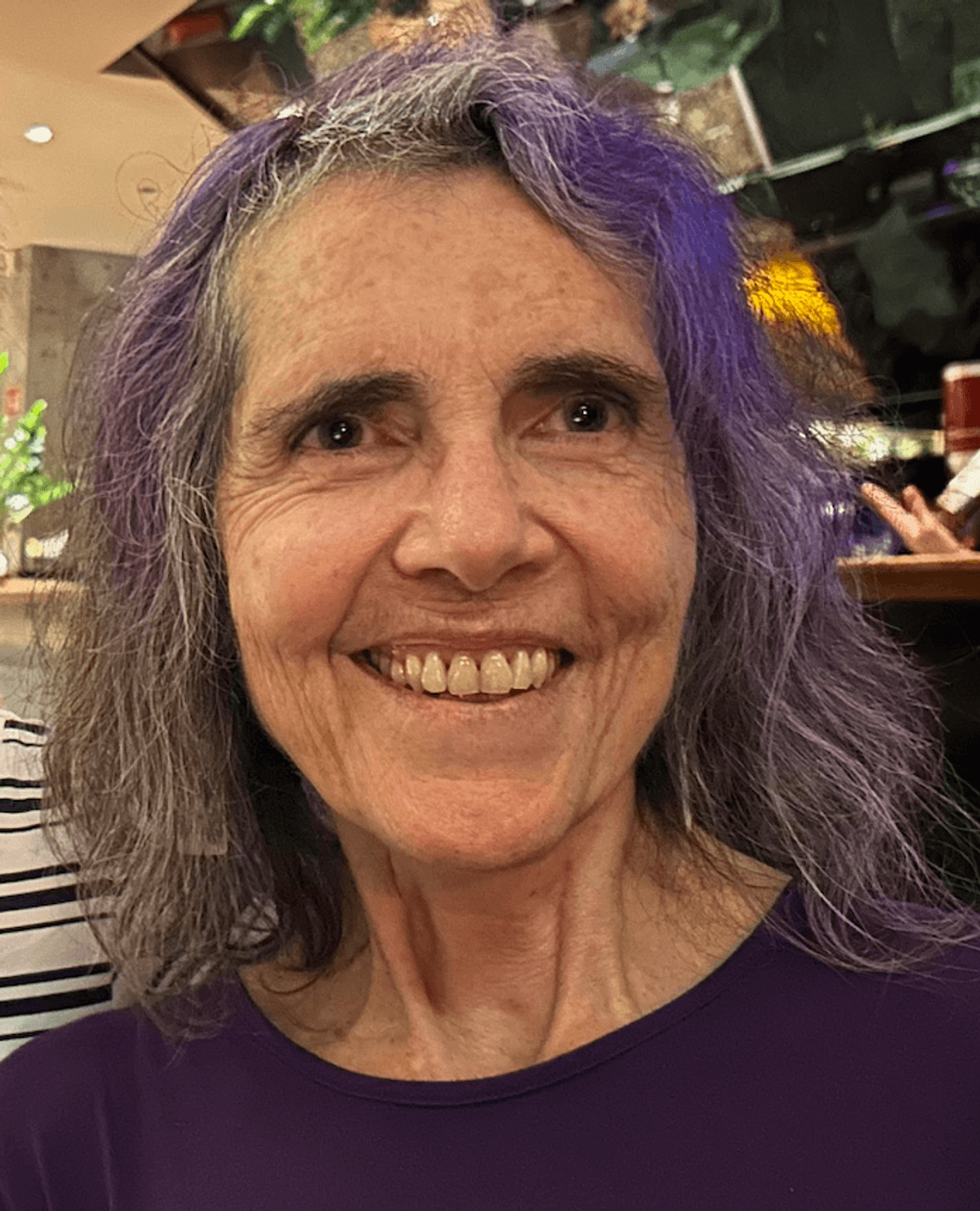
Mycologist Nancy Keller explores fungal genomes for biosynthetic gene clusters that produce novel secondary metabolites.
Nancy Keller
One way that researchers can identify BCGs, said Keller, is to look for genes that encode different types of backbone synthases, which create a “skeleton” on which the rest of the metabolite is built. Other genes in the cluster encode tailoring enzymes, which add elements onto the backbone to create the finished product. BCG atlases can help researchers assess which metabolites are dissimilar from well-characterized natural products, preventing them from wasting time “discovering” the same products over and over again.22
Work by various research groups, including Keller’s, has improved scientific understanding of how BCG expression is regulated, enabling researchers to tinker with fungal genomes to produce greater quantities of the metabolite of interest. If the metabolite comes from a species that is difficult to culture or genetically manipulate, researchers can use fungal artificial chromosomes, a technique developed by Keller in collaboration with scientists at Northwestern University and Intact Genomics, to express the relevant gene cluster in a more cooperative species of fungi.23 The list of potential natural products continues to expand: In 2023, Keller’s team reported on an algorithm that could pick out BCGs with a nontraditional backbone synthase called isocyanide synthase, which revealed thousands of previously unknown BCGs, indicating a massive number of potentially novel metabolites.21
The wealth of new technologies, along with the sheer number of secondary metabolites that that have been unearthed so far, has reinvigorated interest in fungal drug discovery. Unlike the serendipity and secrecy that characterized the discovery and development of penicillin, new antimicrobial agents will likely depend on methodical research and sharing of genomic databases and bioinformatic tools. Similar to the events of nearly a century ago, researchers hope that fungi can once again be enlisted to protect humanity from defeat by deadly pathogens.
- Gaynes R. The discovery of penicillin: New insights after more than 75 years of clinical use.Emerg Infect Dis. 2017;23(5):849.
- Macfarlane G. Alexander Fleming, the man and the myth. Harvard University Press; 1984.
- Fleming A. On the antibacterial action of cultures of a penicillium, with special reference to their use in the isolation of B. influenzae.Br J Exp Pathol. 1929;(10):226-236.
- Alexander Fleming and the discovery of penicillin. In: Germ Theory. John Wiley & Sons, Ltd; 2011:265-293.
- Birkinshaw JH. Harold Raistrick, 1890-1971.Biogr Mem Fellows R Soc. 1997;18:488-509.
- Chain E, et al. Penicillin as a chemotherapeutic agent.Lancet. 1940;236(6104):226-228.
- Abraham EP, et al. Further observations on penicillin.Lancet. 1941;238(6155):177-189.
- Wells PA. Some aspects of the early history of penicillin in the United States.J Wash Acad Sci. 1975;65(3):96-101.
- Neushul P. Science, government and the mass production of penicillin.J Hist Med Allied Sci. 1993;48(4):371-395.
- Raper KB, et al. Penicillin: Natural variation and penicillin production in Penicillium notatum and allied species.J Bacteriol. 1944;48(6):639-659.
- Scoutaris M. “Moldy Mary” and the Illinois fruit and vegetable company.Pharm Hist. 1996;38(4):175-177.
- Gould K. Antibiotics: From prehistory to the present day.J Antimicrob Chemother. 2016;71(3):572-575.
- Waksman SA. Streptomycin: Background, isolation, properties, and utilization.Science. 1953;118(3062):259-266.
- Hutchings MI, et al. Antibiotics: Past, present and future.Curr Opin Microbiol. 2019;51:72-80.
- Naghavi M, et al. Global burden of bacterial antimicrobial resistance 1990–2021: A systematic analysis with forecasts to 2050.Lancet. 2024;404(10459):1199-1226.
- van Rhijn N, et al. Beyond bacteria: The growing threat of antifungal resistance.Lancet. 2024;404(10457):1017-1018.
- Jacobs SE, et al. Candida auris pan-drug-resistant to four classes of antifungal agents. Antimicrob Agents Chemother. 2022;66(7):e0005322.
- Seidel D, et al. Impact of climate change and natural disasters on fungal infections.Lancet Microbe. 2024;5(6):e594-e605.
- Denning DW. Global incidence and mortality of severe fungal disease.Lancet Infect Dis. 2024;24(7):e428-e438.
- Khaldi N, et al. SMURF: Genomic mapping of fungal secondary metabolite clusters.Fungal genet biol. 2010;47(9):736.
- Nickles GR, et al. Mining for a new class of fungal natural products: The evolution, diversity, and distribution of isocyanide synthase biosynthetic gene clusters.Nucleic Acids Res. 2023;51(14):7220-7235.
- Robey MT, et al. An interpreted atlas of biosynthetic gene clusters from 1,000 fungal genomes.PNAS. 2021;118(19):e2020230118.
- Bok JW, et al. Fungal artificial chromosomes for mining of the fungal secondary metabolome.BMC Genom. 2015;16(1):343.